- 1The First Affiliated Hospital of USTC, Division of Life Sciences and Medicine, University of Science and Technology of China, Hefei, China
- 2Turek Clinic, San Francisco, CA, United States
- 3MandelMed, Inc., San Francisco, CA, United States
- 4McLaughlin Research Institute, Touro College of Osteopathic Medicine – Montana (TouroCOM-MT), Great Falls, MT, United States
- 5Research Division, Touro College of Osteopathic Medicine – Montana (TouroCOM-MT), Great Falls, MT, United States
Spermatogonial stem cells (SSCs) are a group of adult stem cells in the testis that serve as the foundation of continuous spermatogenesis and male fertility. SSCs are capable of self-renewal to maintain the stability of the stem cell pool and differentiation to produce mature spermatozoa. Dysfunction of SSCs leads to male infertility. Therefore, dissection of the regulatory network of SSCs is of great significance in understanding the fundamental molecular mechanisms of spermatogonial stem cell function in spermatogenesis and the pathogenesis of male infertility. Furthermore, a better understanding of SSC biology will allow us to culture and differentiate SSCs in vitro, which may provide novel stem cell-based therapy for assisted reproduction. This review summarizes the latest research progress on the regulation of SSCs, and the potential application of SSCs for fertility restoration through in vivo and in vitro spermatogenesis. We anticipate that the knowledge gained will advance the application of SSCs to improve male fertility. Furthermore, in vitro spermatogenesis from SSCs sets the stage for the production of SSCs from induced pluripotent stem cells (iPSCs) and subsequent spermatogenesis.
Introduction
Early in human development, a small group of cells is set aside or allocated to become the germ cells that give rise to the sperm and oocytes that will transmit genetic and epigenetic information to subsequent generations (1). In males, the process of spermatogenesis maintains the production of spermatozoa, the final cell carrier of inheritable material, throughout the lifetime of male mammals (2). Continuous spermatogenesis depends on the appropriate self-renewal and differentiation of spermatogonial stem cells (SSCs) throughout the life of the male (3). The SSCs are the resident stem cell population that resides at the basal membrane of seminiferous tubules of the testis (4, 5). The SSCs can undergo mitotic divisions for self renewal to maintain a steady stem cell pool or they can differentiate through sequential and extensive processes into spermatozoa (6). The balance of self-renewal and differentiation of SSCs is critical, not only for maintaining normal spermatogenesis but also for sustaining lifelong fertility (7). A tilt to self-renewal is a risk factor for germ cell tumors, while a tilt towards differentiation results in exhaustion of germ cell pools, leading to male infertility (8). Numerous studies have demonstrated that the balance between self-renewal and differentiation is precisely controlled by a combination of intrinsic genetic and epigenetic factors within SSCs as well as the extrinsic signals that eminate from the somatic niche (9, 10).
Significantly, SSCs have extraordinary therapeutic potential in assisted reproduction for male infertility (11, 12). Transplantation of SSCs can restore spermatogenesis in patients who suffer from impaired spermatogenesis (13). One application example is fertility preservation of prepubertal boys with cancer and undergoing chemotherapy (14). SSCs can be isolated from testicular biopsy and cryopreserved before chemotherapy, followed by stem cell transplantation into the seminiferous tubules to restore fertility (15, 16). In addition, germline gene therapy using SSCs has been proposed, albeit with obvious concerns regarding legitimate ethical issues, as a promising and feasible approach to treat endocrine disease and metabolic disorders with germline gene mutations (17). Currently, the major hurdle to the use of SSCs in assisted reproductive technology is the difficulty of identificating and isolating endogenous SSCs and directing their differentiation to haploid cells in vitro.
This review provides a brief overview summary of some of the existing knowledge and research progress regarding use of SSCs for inducing spermatogenesis in vivo and in vitro for fertililty restoration. We hope that this summary review may spur further inquiries into details and ongoing studies of practical applications of SSCs in human reproduction and regenerative medicine.
Regulation of SSCS
Human germ cell development begins with the specification of a small group of cells to form the primordial germ cells (PGCs) (18), which are thought to arise from the dorsal amnion at the onset of gastrulation (19). Following their specification, PGCs actively proliferate and migrate to the developing gonad (20–22) where they will occupy the genital ridge and undergo sex-determination by entering either male or female sex-specific developmental pathways (23). External signals from the somatic environment determine the sex of PGCs (24). For male germ cell development, once PGCs occupy the seminiferous tubules of the male gonad, they are termed gonocytes (25), which later interact with the niche cells to become spermatogonia (26). Note that nomenclature is not universal or all inclusive as subtypes exist (example: type A, type b, light and dark spermatogonia), different stages of development are sometimes indicated (examples: early or late spermagonia or undifferentiated and differentiating), or reference to marker content (example: c-kit+ spermatogonia).
The Niche
The architecture of the testes is characterized by two structurally distinct compartments (Figure 1), the seminiferous tubule and the interstitial tissue (27). Within the seminiferous tubule, Sertoli cells form a tight blood-testis barrier to divide the seminiferous epithelium into basal and luminal compartments (28). Developing spermatogonia reside on the basal membrane and are further defined by three types of cells: undifferentiated spermatogonia (quiescent SSCs), differentiating spermatogonia (SSCs that undergo active mitosis), and differentiated spermatogonia (29, 30). The Sertoli cells are the supporting cells for the germ cell population in the testes and are essential for maintaining normal spermatogenesis by providing the cellular matrix and by secreting specific growth factors (31). The surrounding interstitial space consists of various cell types that include the Leydig cells, mesenchymal cells, and immune cells, in addition to lymph vessels, nerve fibers, and connective tissues (27). Leydig cells produce the hormone testosterone and cytokines that may function both directly and indirectly to regulate self-renewal of SSCs (32).
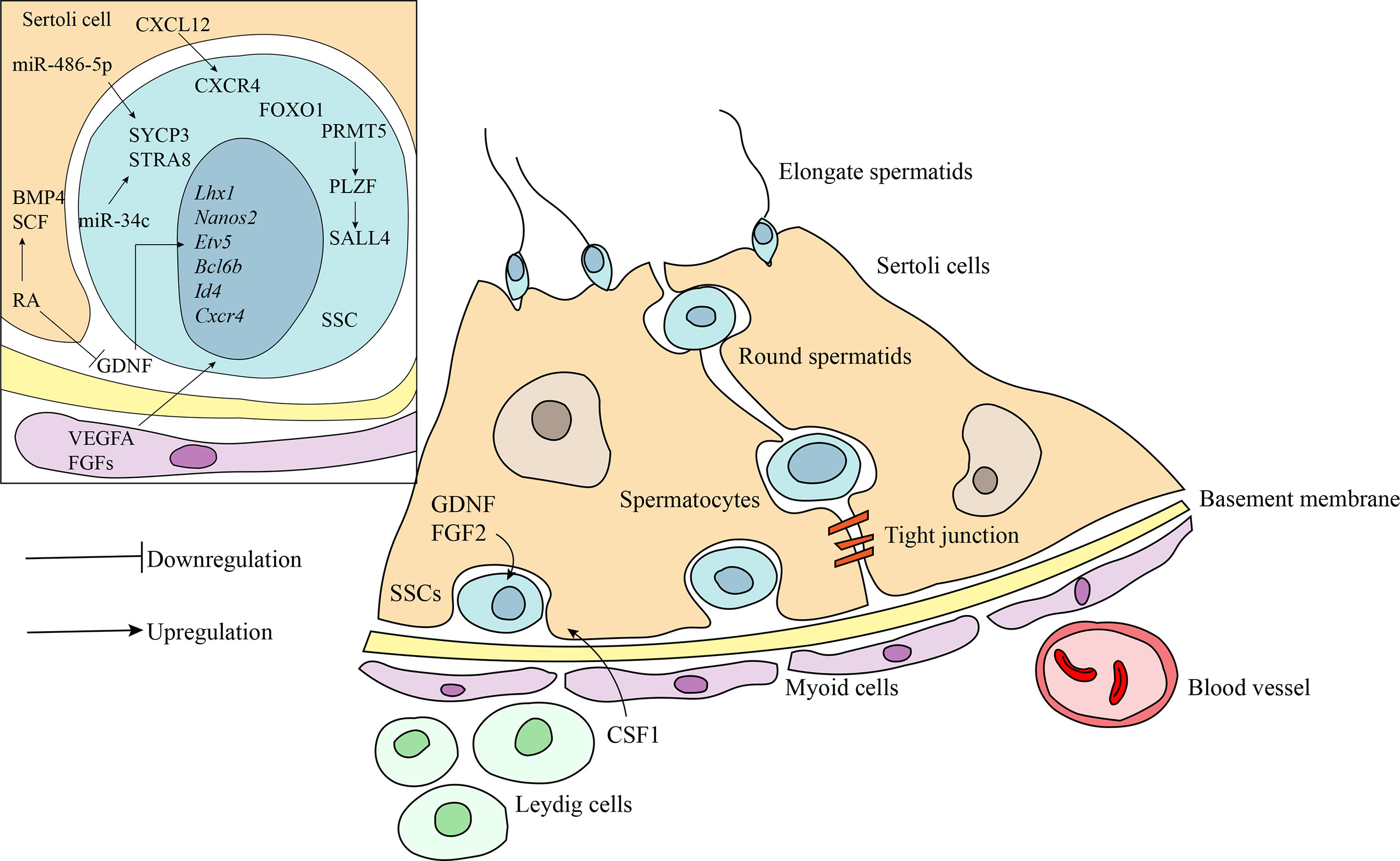
Figure 1 Schematic diagram of the niche of SSCs and the regulatory factors involved in maintaining the stemness and self-renewal of SSCs. Undifferentiated SSCs are localized at the basement membrane. Germ cells maintain the close contact with the Sertoli cells inside the seminiferous epithelium. Peritubular myoid cells surround the seminiferous tubules to form testicular cords. The interstitial compartment consists of many somatic cell types including Leydig cells, mesenchymal cells and immune cells. Bioactive factors in the niche play crucial role in self-renewal and differentiation of SSCs. CXCL12/CXCR4, FGFs, and VEGFA act in synergy with GDNF to maintain SSCs. Retinoic acid (RA) induces the differentiation of SSCs by downregulation, at least in part, of GDNF expression and activation of SCF and BMP4. Transcription factors, PLZF and FOXO1, are involved in regulating SSCs maintenance and spermatogenesis by acting on a subset of downstream target gene. MicroRNAs, including miR-1908-3p, miR-112-5p and miR-31-5p, also act as critical regulators in spermatogenesis.
External and Intrinsic Factors
The fine-tuned balance between self-renewal and differentiation of SSCs is regulated by the interplay of extrinsic and intrinsic factors. GDNF, a growth factor produced by the somatic niche cells, is critical for the maintenance of SSCs both in vivo and in vitro (33). It regulates several essential downstream genes, including the germ cell specific and ubiquitously-expressed genes Nanos2, Etv5, Lhx1, T, Bcl6b, Id1, and Cxcr4, to promote SSC self-renewal and inhibit differentiation (34–39). CXCL12/CXCR4 (39), FGFs (33, 40), and VEGF-A (41) act in synergy with GDNF to maintain SSC stem cell status. In contrast, retinoic acid (RA), a hormone secreted primarily by Sertoli cells, plays an indispensable role in inducing differentiation of SSCs by downregulation of GDNF expression and activation of differentiation-promoting factors, such as BMP and SCF (42–45). Genetic ablation studies in mice indicate that several transcription factors are involved in regulating SSC maintenance and recruitment to spermatogenesis. The PLZF transcription factor is expressed by SSCs and interacts with GDNF signaling as one of the master regulators to promote the self-renewal of SSCs (46, 47). Loss of PLZF results in progressive germ cell loss, testicular hypoplasia, and infertility (46–48). One of the downstream targets of PLZF is the SALL4 protein, which is required for the self-renewal of SSCs and maintenance of ability to enter spermatogenic differentiation (49). A potential upstream regulator of PLZF is PRMT5. Disruption of the PRMT5 gene results in a dramatic reduction of PLZF gene expression, and subsequent progressive loss of SSCs leading to male infertility (50). Another transcription factor important for maintenance of SSC self-renewal is FOXO1, which regulates a number of genes that are preferably expressed in SSCs (51). Deletion of the FOXO1 gene results in defects in SSC maintenance and ultimately spermatogenic failure. In addition, recent research has identified numerous microRNAs as critical regulators in spermatogenesis. Some microRNAs regulate the self-renewal of SSCs. For example, miR-202 plays a crucial role in the maintenance of SSC stemness or self-renewal of the stem cell population (52). Other microRNAs, such as miR-1908-3p (53), miRNA-122-5p (54), and miRNA-31-5p (55), enhance the proliferation and inhibit the early apoptosis of human SSCs via targeting key downstream pathways. Conversely, several microRNAs facilitate differentiation via regulation of the expression of genes associated with SSC differentiation. MiR-34c promotes SSC differentiation by inhibiting the function of the NANOS2 gene, leading to the up-regulation of meiotic-related proteins, STRA8, in mice (56). Similarly, miR-486-5p secreted by Sertoli cells stimulates differentiation of SSCs in mice by up-regulating the expression of STRA8 and SYCP3 (57). Further, impaired spermatogenesis is observed in mice carrying a deficiency in miR-17-92 or a gene deletion of miR-17-92 (58, 59). miR-202 similarly regulates spermatogenesis via orchestration meiotic initiation by preventing precocious differentiation of mouse SSCs (52). Taken together, numerous genes act to balance self-renewal and differentiation of SSCs.
Fertility Restoration Through In Vivo Spermatogenesis
SSCs within the testicular tissues have the potential to complete the entire process of spermatogenesis in vivo and produce functional spermatozoa for fertility restoration (Figure 2). Thus, cryopreservation of testicular tissue prior to gonadotoxic treatment for prepubertal boys is proposed as a helpful strategy for fertility preservation (60). To restore fertility through in vivo spermatogenesis, testicular tissues could be either autotransplanted to the same individual or the tissues might be dissociated to obtain SSCs for autotransplantation. Xenotransplantation would carry the obvious complication of mixing of sperm from different individuals.
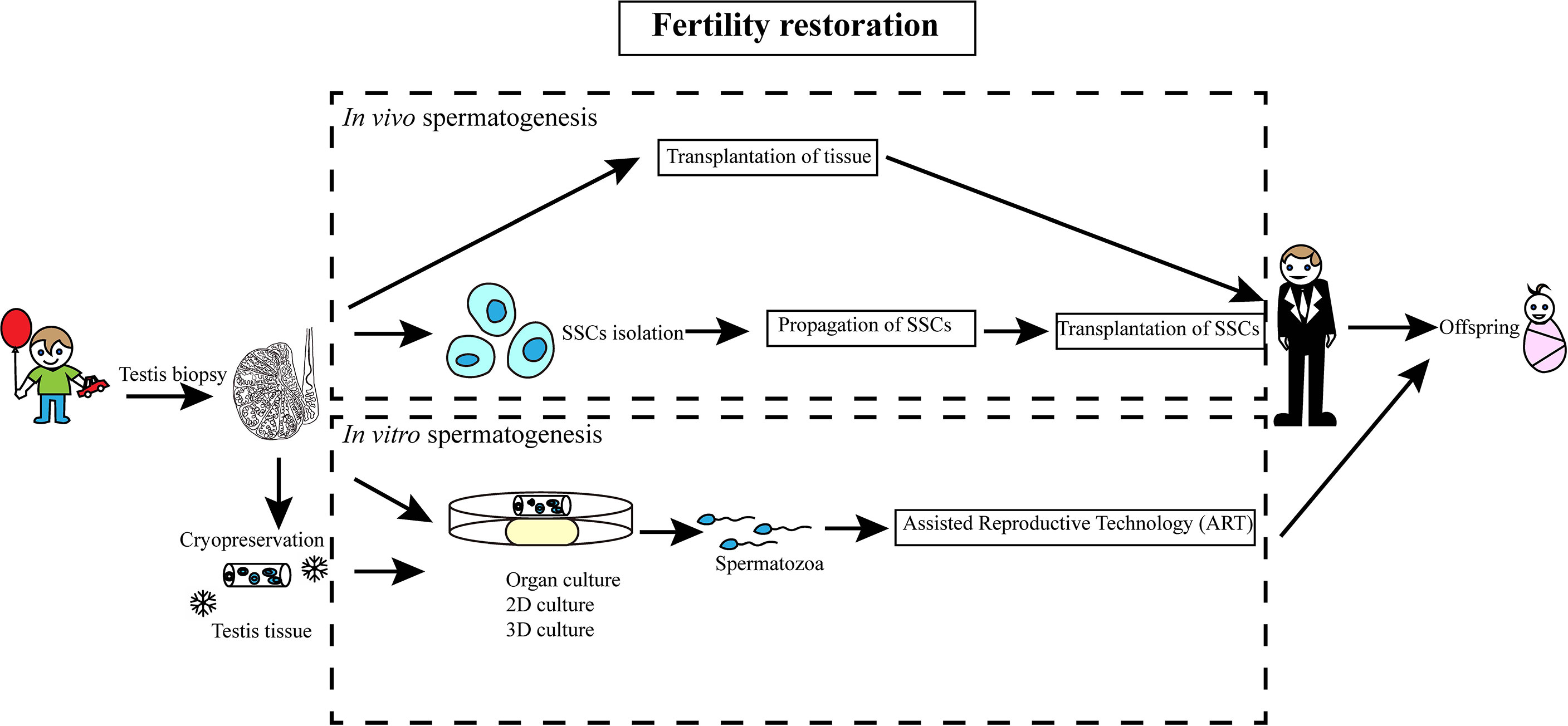
Figure 2 Schematic diagram of SSC-based fertility restoration in humans. A sample of testicular tissue of prepubertal boys, who receive gonadotoxic treatment, is retrieved and cryopreserved. Spermatogenesis may be induced after treatment either in vivo or in vitro.
Transplantation of Testicular Tissues
Autotransplantation of testicular tissues has achieved success in multiple animal models, which results in live offspring (61–65). However, the approach has the risk of re-introducing malignancy is a concern (66). Studies of xenotransplantation, which transplants immature testicular tissue under the back skin of immune-deficient animals, have been used to examine potential complications including malignancy. In 2002, Nagano and colleagues, for example, transplanted human SSCs into immunodeficient mice for the first time (67). Human SSCs survived in mouse testes for at least six months and proliferated during the first month after transplantation.
Transplantation of SSCs
To avoid potential complications of malignancy, isolation of SSCs from cryopreserved testicular tissues followed by transplantation has been proposed as the leading alternative stratgey. To separate SSCs from somatic cells, antibodies that recognize human SSC-specific proteins are used for FACS (fluorescent-activated cell sorting) or MACS (magnetic-activated cell sorting) for sorting SSCs from other cell types. Antibodies that have been shown to be useful for sorting SSCs include GFRα (68), GPR125, ID4 (69), ITGA6 (70), SSEA4 (71), PLPPR3 (72), and OCT4 (73). An alternative to cell sorting is to take advantage of different physical properties between SSCs and somatic cells such as velocity sedimentation and differential affinity to extracellular matrices on the culture plate (74–78). Once isolated, SSCs are cultured with growth factors shown to be optimal or essential for SSC maintenance [GDNF, BFGF, EGF, and LIF (79–81)].
A major limitation of SSC transplantation in vivo, for fertility restoration in clinical practice, is the scarcity of SSCs within the testicular tissue. This has necessitated exploration of alternatives including the establishment of a robust in vitro culture system to maintain and expand human SSCs. Extensive effort has been focused on optimization of culture conditions for long-term maintenance and propagation of human SSCs. Multiple culture substrates, including hydrogel, matrigel, and laminin, have been shown to promote the propagation of human SSCs under feeder-free conditions (82). Currently, several markers are used for the verification of human SSCs. However, many of these markers are also expressed in testicular somatic cells. For example, UCHL1, which was used to identify SSCs from humans, is also expressed in Leydig cells and nerve fibers (83). The most stringent assay to assess the function of SSCs is to generate offspring after homologous transplantation. However, despite success in animal models, including non-human primates, no studies are reporting the generation of human functional spermatozoa following autotransplantation or xenotransplantation of testicular tissue or isolated human SSCs for fertility restoration.
Fertility Restoration Through In Vitro Spermatogenesis
The establishment of a system to recapitulate spermatogenesis and generate spermatozoa in vitro can not only be directly applied in assisted reproduction, such as in vitro fertilization (IVF) or intracytoplasmic sperm injection (ICSI), but also provide a convenient system to study the molecular mechanisms and genetic causes for male infertility. Building a functional somatic microenvironment is critical for in vitro spermatogenesis. Several strategies, including exploitation of intrinsic somatic microenvironment by organotypic culture, two-dimensional culture, and three-dimensional culture of testis cell suspensions.
Organotypic Culture
Since 1959, a gas-liquid interface was used to culture testicular fragments of the adult rats (84). In this culture system, the differentiation of SSCs was limited up to pachytene spermatocytes (85). In 2003, round spermatids were observed after two weeks of culture in a gas-liquid interface culture system (86). Several other organotypic culture systems have been developed to recapitulate the entire process of spermatogenesis in vitro. One of the breakthroughs in the research was reported in 2011 with the demonstration of live offspring that were generated from in vitro-produced haploid germ cells (87). In this study, testicular tissue fragments from neonatal mice were cultured on an agarose gel-based organ culture system. Subsequently, microfluidic technology was adopted for organ culture, with the goal of providing a better culture environment for SSCs by facilitating the exchange of gases, nutrients, and waste products (88). Recently, successful recapitulation of human testicular organogenesis from fetal gonads was achieved, and in vitro-derived haploid spermatids were shown to undergo meiotic recombination (89).
Two-Dimensional Culture
2D culture systems with testis cell suspensions have been widely used for SSC proliferation and differentiation with two primary types of 2D culture systems most common: (1) SSCs cultured on mitotically-inactivated feeder cells, (2) SSCs co-cultured with somatic cells (90). Using the support of 2D culture sytems, numerous studies have reported that haploid male germ cells could be induced (91–95), and offspring can be produced from these in vitro derived haploid male germ cells in rodent (96). However, the 2D culture system has not been optimized for human germ cells. This may be due to the lack of spatial structure of seminiferous tubules and proper interactions between germ cells and somatic cells.
Three-Dimensional Culture
To better mimic the testicular niche, various 3D culture systems have been developed. In 2006, testicular cells isolated from rats were cultured on collagen gels to mimic the composition of the basal membrane of seminiferous tubules (97). Later, the soft-agar culture system (SACS) was developed (98), and mice haploid germ cells from undifferentiated germ cells were generated in this system in 2012 (99). The SACS system also supports the differentiiation of SSCs of non-human primates. The most commonly used alternate material in 3D culture system is methylcellulose. The methylcellulose culture system (MCS) also supports the differentiation of immature germ cells.
In order to artificially reproduce the in vivo form and function of the seminiferous epithelium, a 3D engineered blood-testis barrier (eBTB) system was designed in 2010 (100). Testicular peritubular myoid cells were first cultured on the underside of culture inserts, and then germ cells and Sertoli cells were added on top of the inserts. The testicular cells from neonatal mice form the aggregate by culturing on a V-shaped plate. The aggregate plated on the top of agarose gel blocks, and the haploid male germ cells were obtained after 30-51 days of incubation (101).
The 3D decellularized testicular scaffold with hyaluronic acid and chitosan provides the condition for the differentiation and proliferation of mice SSCs (102). The proliferation and self-renewal of mice SSCs was stimulated by culturing on the 3D scaffold consisting of alginate hydrogel with Sertoli cells (103). The mice germ cells were cultured in 3D printed one-layer scaffolds at the air-medium interface simulating the tubule-like structure. This culture system provided the condition for long-term survival and differentiation (104).
Soft agar and agarose gel are the most common material used to establish the 3D culture system for human SSCs. A soft agar culture system has been shown to support the proliferation and differentiation of human SSCs (105). Another material that has been used in 3D culture systems for human SSCs is a polycaprolactone (PCL) nanofiber matrix (106). This material may mimic the physical form of collagen fibers in the natural extracellular matrix (107).
Conclusion and Perspectives
With the development of technologies, including -omics at the single-cell level, lineage-tracing, spermatogonial transplantation, and in vitro culturing and differentiation, we start decoding the secrets of SSCs. However, the application of SSCs to treat male infertility necessitates extensive studies to ensure safety and efficacy. An efficient culture condition for human SSCs to ensure their propagation, as well as proper animal models for xenotransplantation, will assist in assessing safety and efficacy as indicated by recent studies (108). Furthermore, establishing a robust system for in vitro spermatogenesis is also helpful for pharmaceutical or toxicological studies for new drugs. Finally, in vitro spermatogenesis from SSCs sets the stage for the production of SSCs from induced pluripotent stem cells (iPSCs) and subsequent spermatogenesis. For example, studies are underway to integrate data and practices from divergent fields to promote spermatogenesis from iPSCs via co-culture with Sertoli cells in a 2D-, 3D- or a modified environment, similar to those used in other physiological systems, that might more faithfully mimic spermatogenic dynamics including circulation (109, 110).
Author Contributions
All authors contributed to the article and approved the submitted version.
Funding
This work was supported by an NIH grant to RRP #HD096026 and National Natural Science Foundation of China #32070830 to FF.
Conflict of Interest
Authors PJT and CMJ are founders of the company MandelMed. No funding from MandelMed is associated with this study.
The remaining authors declare that the research was conducted in the absence of any commercial or financial relationships that could be construed as a potential conflict of interest.
Publisher’s Note
All claims expressed in this article are solely those of the authors and do not necessarily represent those of their affiliated organizations, or those of the publisher, the editors and the reviewers. Any product that may be evaluated in this article, or claim that may be made by its manufacturer, is not guaranteed or endorsed by the publisher.
References
1. Reik W, Surani MA. Germline and Pluripotent Stem Cells. Cold Spring Harbor Perspect Biol (2015) 7:a019422.
2. Xi HM, Ren YJ, Ren F, Li Y, Feng TY, Wang Z, et al. Recent Advances in Isolation, Identification, and Culture of Mammalian Spermatogonial Stem Cells. Asian J Androl (2022) 24:5–14.
3. Chen SR, Liu YX. Regulation of Spermatogonial Stem Cell Self-Renewal and Spermatocyte Meiosis by Sertoli Cell Signaling. Reproduction (2015) 149:R159–67.
4. Lei Q, Hamer G. The Use of Spermatogonial Stem Cells to Correct a Mutation Causing Meiotic Arrest. Asian J Androl (2021) 23:600–1.
5. Wang J, Liu C, Fujino M, Tong G, Zhang Q, Li XK, et al. Stem Cells as a Resource for Treatment of Infertility-Related Diseases. Curr Mol Med (2019) 19:539–46.
6. Zhao X, Wan W, Zhang X, Wu Z, Yang H. Spermatogonial Stem Cell Transplantation in Large Animals. Animals (2021) 11:918.
7. Alpaugh WF, Voigt AL, Dardari R, Su L, Al Khatib I, Shin W, et al. Loss of Ubiquitin Carboxy-Terminal Hydrolase L1 Impairs Long-Term Differentiation Competence and Metabolic Regulation in Murine Spermatogonial Stem Cells. Cells (2021) 10:2265.
8. Singh SR, Burnicka-Turek O, Chauhan C, Hou SX. Spermatogonial Stem Cells, Infertility and Testicular Cancer. J Cell Mol Med (2011) 15:468–83.
9. Jones DL, Wagers AJ. No Place Like Home: Anatomy and Function of the Stem Cell Niche. Mol Cell Biol (2008) 9:11–21.
10. La HM, Hobbs RM. Mechanisms Regulating Mammalian Spermatogenesis and Fertility Recovery Following Germ Cell Depletion. Cell Mol Life Sci (2019) 76:4071–102.
11. Hermann BP, Sukhwani M, Winkler F, Pascarella JN, Peters KA, Sheng Y, et al. Spermatogonial Stem Cell Transplantation Into Rhesus Testes Regenerates Spermatogenesis Producing Functional Sperm. Cell Stem Cell (2012) 11:715–26.
12. Forbes CM, Flannigan R, Schlegel PN. Spermatogonial Stem Cell Transplantation and Male Infertility: Current Status and Future Directions. Arab J Urol (2018) 16:171–80.
13. Kanatsu-Shinohara M, Toyokuni S, Morimoto T, Matsui S, Honjo T, Shinohara T. Functional Assessment of Self-Renewal Activity of Male Germline Stem Cells Following Cytotoxic Damage and Serial Transplantation. Biol Reprod (2003) 68:1801–7.
14. Gul M, Hildorf S, Dong L, Thorup J, Hoffmann ER, Jensen CFS, et al. Review of Injection Techniques for Spermatogonial Stem Cell Transplantation. Hum Reprod Update (2020) 26:368–91.
15. Levine JM. Preserving Fertility in Children and Adolescents With Cancer. Children (2014) 1:166–85.
16. Onofre J, Baert Y, Faes K, Goossens E. Cryopreservation of Testicular Tissue or Testicular Cell Suspensions: A Pivotal Step in Fertility Preservation. Hum Reprod Update (2016) 22:744–61.
17. Kubota H, Brinster RL. Technology Insight: In Vitro Culture of Spermatogonial Stem Cells and Their Potential Therapeutic Uses. Endocrinol Metab (2006) 2:99–108.
18. von Meyenn F, Reik W. Forget the Parents: Epigenetic Reprogramming in Human Germ Cells. Cell (2015) 161:1248–51.
19. Sasaki K, Nakamura T, Okamoto I, Yabuta Y, Iwatani C, Tsuchiya H, et al. The Germ Cell Fate of Cynomolgus Monkeys is Specified in the Nascent Amnion. Dev Cell (2016) 39:169–85.
20. Santos AC, Lehmann R. Germ Cell Specification and Migration in Drosophila and Beyond. Curr Biol (2004) 14:R578–89.
21. Chen SR, Zheng QS, Zhang Y, Gao F, Liu YX. Disruption of Genital Ridge Development Causes Aberrant Primordial Germ Cell Proliferation But Does Not Affect Their Directional Migration. BMC Biol (2013) 11:22.
22. Updike DL. Quelling Germ Cell Pluripotency on the Genital Ridge. Proc Natl Acad Sci (2019) 116:25374–5.
23. Larose H, Shami AN, Abbott H, Manske G, Lei L, Hammoud SS. Gametogenesis: A Journey From Inception to Conception. Curr Top Dev Biol (2019) 132:257–310.
24. Murray SM, Yang SY, Van Doren M. Germ Cell Sex Determination: A Collaboration Between Soma and Germline. Curr Opin Cell Biol (2010) 22:722–9.
25. Culty M. Gonocytes, the Forgotten Cells of the Germ Cell Lineage. Birth Def Res Part C Embryo Today (2009) 87:1–26.
26. Ma F, Wang X, Chung SSW, Sicinski P, Shang E, Wolgemuth DJ. Cyclin A2 Is Essential for Mouse Gonocyte Maturation. Cell Cycle (2020) 19:1654–64.
27. Oatley JM, Brinster RL. The Germline Stem Cell Niche Unit in Mammalian Testes. Phys Rev (2012) 92:577–95.
28. Ogawa T, Ohmura M, Ohbo K. The Niche for Spermatogonial Stem Cells in the Mammalian Testis. Intl J Hematol (2005) 82:381–8.
29. Rowley MJ, Berlin JD, Heller CG. The Ultrastructure of Four Types of Human Spermatogonia. Zellforsch Mikrosk Ana (1971) 112:139–57.
31. Griswold MD. The Central Role of Sertoli Cells in Spermatogenesis. Sem Cell Dev Biol (1998) 9:411–6.
32. Zaker H, Razi M, Mahmoudian A, Soltanalinejad F. Boosting Effect of Testosterone on GDNF Expression in Sertoli Cell Line (TM4): Comparison Between TM3 Cells-Produced and Exogenous Testosterone. Gene (2022) 812:146112.
33. Kubota H, Avarbock MR, Brinster RL. Growth Factors Essential for Self-Renewal and Expansion of Mouse Spermatogonial Stem Cells. Proc Natl Acad Sci (2004) 101:16489–94.
34. Sada A, Hasegawa K, Pin PH, Saga Y. NANOS2 Acts Downstream of Glial Cell Line-Derived Neurotrophic Factor Signaling to Suppress Differentiation of Spermatogonial Stem Cells. Stem Cells (2012) 30:280–91.
35. Chen C, Ouyang W, Grigura V, Zhou Q, Carnes K, Lim H, et al. ERM is Required for Transcriptional Control of the Spermatogonial Stem Cell Niche. Nature (2005) 436:1030–4.
36. Oatley JM, Avarbock MR, Telaranta AI, Fearon DT, Brinster RL. Identifying Genes Important for Spermatogonial Stem Cell Self-Renewal and Survival. Proc Natl Acad Sci (2006) 103:9524–9.
37. Oatley JM, Avarbock MR, Brinster RL. Glial Cell Line-Derived Neurotrophic Factor Regulation of Genes Essential for Self-Renewal of Mouse Spermatogonial Stem Cells is Dependent on Src Family Kinase Signaling. J Biol Chem (2007) 282:25842–51.
38. Oatley MJ, Kaucher AV, Racicot KE, Oatley JM. Inhibitor of DNA Binding 4 is Expressed Selectively by Single Spermatogonia in the Male Germline and Regulates the Self-Renewal of Spermatogonial Stem Cells in Mice. Biol Reprod (2011) 85:347–56.
39. Yang QE, Kim D, Kaucher A, Oatley MJ, Oatley JM. CXCL12-CXCR4 Signaling is Required for the Maintenance of Mouse Spermatogonial Stem Cells. J Cell Sci (2013) 126:1009–20.
40. Mirzapour T, Movahedin M, Tengku Ibrahim TA, Koruji M, Haron AW, Nowroozi MR, et al. Effects of Basic Fibroblast Growth Factor and Leukaemia Inhibitory Factor on Proliferation and Short-Term Culture of Human Spermatogonial Stem Cells. Andrologia (2012) 44S:41–55.
41. Caires KC, de Avila JM, Cupp AS, McLean DJ. VEGFA Family Isoforms Regulate Spermatogonial Stem Cell Homeostasis in vivo. Endocrinol (2012) 153:887–900.
42. Pellegrini M, Filipponi D, Gori M, Barrios F, Lolicato F, Grimaldi P, et al. ATRA and KL Promote Differentiation Toward the Meiotic Program of Male Germ Cells. Cell Cycle (2008) 7:3878–88.
43. Carlomagno G, van Bragt MP, Korver CM, Repping S, de Rooij DG, van Pelt AM. BMP4-Induced Differentiation of a Rat Spermatogonial Stem Cell Line Causes Changes in its Cell Adhesion Properties. Biol Reprod (2010) 83:742–9.
44. Barrios F, Filipponi D, Campolo F, Gori M, Bramucci F, Pellegrini M, et al. SOHLH1 and SOHLH2 Control Kit Expression During Postnatal Male Germ Cell Development. J Cell Sci (2012) 125:1455–64.
45. Yang QE, Racicot KE, Kaucher AV, Oatley MJ, Oatley JM. MicroRNAs 221 and 222 Regulate the Undifferentiated State in Mammalian Male Germ Cells. Development (2013) 140:280–90.
46. Costoya JA, Hobbs RM, Barna M, Cattoretti G, Manova K, Sukhwani M, et al. Essential Role of Plzf in Maintenance of Spermatogonial Stem Cells. Nat Genet (2004) 36:653–9.
47. Buaas FW, Kirsh AL, Sharma M, McLean DJ, Morris JL, Griswold MD, et al. Plzf is Required in Adult Male Germ Cells for Stem Cell Self-Renewal. Nat Genet (2004) 36:647–52.
48. Fischer S, Kohlhase J, Böhm D, Schweiger B, Hoffmann D, Heitmann M, et al. Biallelic Loss of Function of the Promyelocytic Leukaemia Zinc Finger (PLZF) Gene Causes Severe Skeletal Defects and Genital Hypoplasia. J Med Genet (2008) 45:731–7.
49. Hobbs RM, Fagoonee S, Papa A, Webster K, Altruda F, Nishinakamura R, et al. Functional Antagonism Between Sall4 and Plzf Defines Germline Progenitors. Cell Stem Cell (2012) 10:284–98.
50. Dong F, Chen M, Chen M, Jiang L, Shen Z, Ma L, et al. PRMT5 is Involved in Spermatogonial Stem Cells Maintenance by Regulating Plzf Expression via Modulation of Lysine Histone Modifications. Front Cell Dev Biol (2021) 9:673258.
51. Goertz MJ, Wu Z, Gallardo TD, Hamra FK, Castrillon DH. Foxo1 is Required in Mouse Spermatogonial Stem Cells for Their Maintenance and the Initiation of Spermatogenesis. J Clin Invest (2011) 121:3456–66.
52. Chen J, Gao C, Lin X, Ning Y, He W, Zheng C, et al. The microRNA miR-202 Prevents Precocious Spermatogonial Differentiation and Meiotic Initiation During Mouse Spermatogenesis. Development (2021) 148:199799.
53. Chen W, Cui Y, Liu B, Li C, Du L, Tang R, et al. Hsa-miR-1908-3p Mediates the Self-Renewal and Apoptosis of Human Spermatogonial Stem Cells via Targeting KLF2. Nucl Acids (2020) 20:788–800.
54. Zhou F, Chen W, Cui Y, Liu B, Yuan Q, Li Z, et al. miRNA-122-5p Stimulates the Proliferation and DNA Synthesis and Inhibits the Early Apoptosis of Human Spermatogonial Stem Cells by Targeting CBL and Competing With lncRNA Casc7. Aging (2020) 12:25528–46.
55. Fu H, Zhou F, Yuan Q, Zhang W, Qiu Q, Yu X, et al. miRNA-31-5p Mediates the Proliferation and Apoptosis of Human Spermatogonial Stem Cells via Targeting JAZF1 and Cyclin A2. Nucl Acids (2019) 14:90–100.
56. Yu M, Mu H, Niu Z, Chu Z, Zhu H, Hua J. miR-34c Enhances Mouse Spermatogonial Stem Cells Differentiation by Targeting Nanos2. J Cell Biochem (2014) 115:232–42.
57. Li Q, Li H, Liang J, Mei J, Cao Z, Zhang L, et al. Sertoli Cell-Derived Exosomal MicroRNA-486-5p Regulates Differentiation of Spermatogonial Stem Cell Through PTEN in Mice. J Cell Mol Med (2021) 25:3950–62.
58. Tong MH, Mitchell DA, McGowan SD, Evanoff R, Griswold MD. Two miRNA Clusters, Mir-17-92 (Mirc1) and Mir-106b-25 (Mirc3), are Involved in the Regulation of Spermatogonial Differentiation in Mice. Biol Reprod (2012) 86:72.
59. Xie R, Lin X, Du T, Xu K, Shen H, Wei F, et al. Targeted Disruption of miR-17-92 Impairs Mouse Spermatogenesis by Activating mTOR Signaling Pathway. Medicine (2016) 95:e2713.
60. Picton HM, Wyns C, Anderson RA, Goossens E, Jahnukainen K, Kliesch S, et al. A European Perspective on Testicular Tissue Cryopreservation for Fertility Preservation in Prepubertal and Adolescent Boys. Hum Reprod (2015) 30:2463–75.
61. Brinster RL, Avarbock MR. Germline Transmission of Donor Haplotype Following Spermatogonial Transplantation. Proc Natl Acad Sci (1994) 91:11303–7.
62. Li CH, Yan LZ, Ban WZ, Tu Q, Wu Y, Wang L, et al. Long-Term Propagation of Tree Shrew Spermatogonial Stem Cells in Culture and Successful Generation of Transgenic Offspring. Cell Res (2017) 27:241–52.
63. Honaramooz A, Behboodi E, Megee SO, Overton SA, Galantino-Homer H, Echelard Y, et al. Fertility and Germline Transmission of Donor Haplotype Following Germ Cell Transplantation in Immunocompetent Goats. Biol Reprod (2003) 69:1260–4.
64. Herrid M, Olejnik J, Jackson M, Suchowerska N, Stockwell S, Davey R, et al. Irradiation Enhances the Efficiency of Testicular Germ Cell Transplantation in Sheep. Biol Reprod (2009) 81:898–905.
65. Ryu BY, Orwig KE, Avarbock MR, Brinster RL. Stem Cell and Niche Development in the Postnatal Rat Testis. Dev Biol (2003) 263:253–63.
66. Lee J, Shinohara T. Epigenetic Modifications and Self-Renewal Regulation of Mouse Germline Stem Cells. Cell Res (2011) 21:1164–71.
67. Nagano M, Patrizio P, Brinster RL. Long-Term Survival of Human Spermatogonial Stem Cells in Mouse Testes. Fertil Steril (2002) 78:1225–33.
68. He Z, Kokkinaki M, Jiang J, Dobrinski I, Dym M. Isolation, Characterization, and Culture of Human Spermatogonia. Biol Reprod (2010) 82:363–72.
69. Sachs C, Robinson BD, Andres Martin L, Webster T, Gilbert M, Lo HY, et al. Evaluation of Candidate Spermatogonial Markers ID4 and GPR125 in Testes of Adult Human Cadaveric Organ Donors. Androl (2014) 2:607–14.
70. Valli H, Sukhwani M, Dovey SL, Peters KA, Donohue J, Castro CA, et al. Fluorescence- and Magnetic-Activated Cell Sorting Strategies to Isolate and Enrich Human Spermatogonial Stem Cells. Fertil Steril (2014) 102:566–80.
71. Altman E, Yango P, Moustafa R, Smith JF, Klatsky PC, Tran ND. Characterization of Human Spermatogonial Stem Cell Markers in Fetal, Pediatric, and Adult Testicular Tissues. Reproduction (2014) 148:417–27.
72. Tan K, Song HW, Thompson M, Munyoki S, Sukhwani M, Hsieh TC, et al. Transcriptome Profiling Reveals Signaling Conditions Dictating Human Spermatogonia Fate in vitro. Proc Natl Acad Sci (2020) 117:17832–41.
73. Bhartiya D, Kasiviswanathan S, Unni SK, Pethe P, Dhabalia JV, Patwardhan S, et al. Newer Insights Into Premeiotic Development of Germ Cells in Adult Human Testis Using Oct-4 as a Stem Cell Marker. J Histochem Cytochem (2010) 58:1093–106.
74. Koh KB, Komiyama M, Toyama Y, Adachi T, Mori C. Percoll Fractionation of Adult Mouse Spermatogonia Improves Germ Cell Transplantation. Asian J Androl (2004) 6:93–8.
75. Bucci LR, Brock WA, Johnson TS, Meistrich ML. Isolation and Biochemical Studies of Enriched Populations of Spermatogonia and Early Primary Spermatocytes From Rat Testes. Biol Reprod (1986) 34:195–206.
76. Izadyar F, Spierenberg GT, Creemers LB, den Ouden K, de Rooij DG. Isolation and Purification of Type A Spermatogonia From the Bovine Testis. Reproduction (2002) 124:85–94.
77. Rodriguez-Sosa JR, Dobson H, Hahnel A. Isolation and Transplantation of Spermatogonia in Sheep. Theriogenology (2006) 66:2091–103.
78. Liu S, Tang Z, Xiong T, Tang W. Isolation and Characterization of Human Spermatogonial Stem Cells. Reprod Biol Endocrinol (2011) 9:141.
79. Martin LA, Seandel M. Propagation of Adult SSCs: From Mouse to Human. BioMed Res Intl (2013) 4:384734.
80. Kanatsu-Shinohara M, Inoue K, Ogonuki N, Miki H, Yoshida S, Toyokuni S, et al. Leukemia Inhibitory Factor Enhances Formation of Germ Cell Colonies in Neonatal Mouse Testis Culture. Biol Reprod (2007) 76:55–62.
81. Wang P, Suo LJ, Wang YF, Shang H, Li GX, Hu JH, et al. Effects of GDNF and LIF on Mouse Spermatogonial Stem Cells Proliferation in vitro. Cytotechnol (2014) 66:309–16.
82. Martin-Inaraja M, Ferreira M, Taelman J, Eguizabal C, Chuva De Sousa Lopes SM. Improving In Vitro Culture of Human Male Fetal Germ Cells. Cells (2021) 10:2033.
83. von Kopylow K, Kirchhoff C, Jezek D, Schulze W, Feig C, Primig M, et al. Screening for Biomarkers of Spermatogonia Within the Human Testis: A Whole Genome Approach. Hum Reprod (2010) 25:1104–12.
85. Steinberger A, Steinberger E. Stimulatory Effect of Vitamins and Glutamine on the Differentiation of Germ Cells in Rat Testes Organ Culture Grown in Chemically Defined Media. Exp Cell Res (1966) 44:429–35.
86. Suzuki S, Sato K. The Fertilising Ability of Spermatogenic Cells Derived From Cultured Mouse Immature Testicular Tissue. Zygote (2003) 11:307–16.
87. Sato T, Katagiri K, Gohbara A, Inoue K, Ogonuki N, Ogura A, et al. In Vitro Production of Functional Sperm in Cultured Neonatal Mouse Testes. Nature (2011) 471:504–7.
88. Komeya M, Kimura H, Nakamura H, Yokonishi T, Sato T, Kojima K, et al. Long-Term Ex Vivo Maintenance of Testis Tissues Producing Fertile Sperm in a Microfluidic Device. Sci Rep (2016) 6:21472.
89. Yuan Y, Li L, Cheng Q, Diao F, Zeng Q, Yang X, et al. In Vitro Testicular Organogenesis From Human Fetal Gonads Produces Fertilization-Competent Spermatids. Cell Res (2020) 30:244–55.
90. Ibtisham F, Honaramooz A. Spermatogonial Stem Cells for In Vitro Spermatogenesis and In Vivo Restoration of Fertility. Cells (2020) 9:745.
91. Nagao Y. Viability of Meiotic Prophase Spermatocytes of Rats is Facilitated in Primary Culture of Dispersed Testicular Cells on Collagen Gel by Supplementing Epinephrine or Norepinephrine: Evidence That Meiotic Prophase Spermatocytes Complete Meiotic Divisions in vitro. In Vitro Cell Dev Biol (1989) 25:1088–98.
92. Rassoulzadegan M, Paquis-Flucklinger V, Bertino B, Sage J, Jasin M, Miyagawa K, et al. Transmeiotic Differentiation of Male Germ Cells in Culture. Cell (1993) 75:997–1006.
93. Hue D, Staub C, Perrard-Sapori MH, Weiss M, Nicolle JC, Vigier M, et al. Meiotic Differentiation of Germinal Cells in Three-Week Cultures of Whole Cell Population From Rat Seminiferous Tubules. Biol Reprod (1998) 59:379–87.
94. Staub C, Hue D, Nicolle JC, Perrard-Sapori MH, Segretain D, Durand P. The Whole Meiotic Process can Occur In Vitro in Untransformed Rat Spermatogenic Cells. Exp Cell Res (2000) 260:85–95.
95. Wang P, Suo LJ, Shang H, Li Y, Li GX, Li QW, et al. Differentiation of Spermatogonial Stem Cell-Like Cells From Murine Testicular Tissue Into Haploid Male Germ Cells in vitro. Cytotechnol (2014) 66:365–72.
96. Marh J, Tres LL, Yamazaki Y, Yanagimachi R, Kierszenbaum AL. Mouse Round Spermatids Developed In Vitro From Preexisting Spermatocytes can Produce Normal Offspring by Nuclear Injection Into In Vivo-Developed Mature Oocytes. Biol Reprod (2003) 69:169–76.
97. Lee JH, Kim HJ, Kim H, Lee SJ, Gye MC. In Vitro Spermatogenesis by Three-Dimensional Culture of Rat Testicular Cells in Collagen Gel Matrix. Biomaterials (2006) 27:2845–53.
98. Stukenborg JB, Wistuba J, Luetjens CM, Elhija MA, Huleihel M, Lunenfeld E, et al. Coculture of Spermatogonia With Somatic Cells in a Novel Three-Dimensional Soft-Agar-Culture-System. J Androl (2008) 29:312–29.
99. Abu Elhija M, Lunenfeld E, Schlatt S, Huleihel M. Differentiation of Murine Male Germ Cells to Spermatozoa in a Soft Agar Culture System. Asian J Androl (2012) 14:285–93.
100. Legendre A, Froment P, Desmots S, Lecomte A, Habert R, Lemazurier E. An Engineered 3D Blood-Testis Barrier Model for the Assessment of Reproductive Toxicity Potential. Biomaterials (2010) 31:4492–505.
101. Yokonishi T, Sato T, Katagiri K, Komeya M, Kubota Y, Ogawa T. In Vitro Reconstruction of Mouse Seminiferous Tubules Supporting Germ Cell Differentiation. Biol Reprod (2013) 89:15.
102. Naeemi S, Eidi A, Khanbabaee R, Sadri-Ardekani H, Kajbafzadeh AM. Differentiation and Proliferation of Spermatogonial Stem Cells Using a Three-Dimensional Decellularized Testicular Scaffold: A New Method to Study the Testicular Microenvironment In Vitro. Intl Urol Nephrol (2021) 53:1543–50.
103. Veisi M, Mansouri K, Assadollahi V, Jalili C, Pirnia A, Salahshoor MR, et al. Evaluation of Co-Cultured Spermatogonial Stem Cells Encapsulated in Alginate Hydrogel With Sertoli Cells and Their Transplantation Into Azoospermic Mice. Zygote (2021) 1–8. doi: 10.1017/S0967199421000733
104. Richer G, Hobbs RM, Loveland KL, Goossens E, Baert Y. Long-Term Maintenance and Meiotic Entry of Early Germ Cells in Murine Testicular Organoids Functionalized by 3D Printed Scaffolds and Air-Medium Interface Cultivation. Front Physiol (2021) 12:757565.
105. Mohammadzadeh E, Mirzapour T, Nowroozi MR, Nazarian H, Piryaei A, Alipour F, et al. Differentiation of Spermatogonial Stem Cells by Soft Agar Three-Dimensional Culture System. Artif Cells Nanomed Biotechnol (2019) 47:1772–81.
106. Bashiri Z, Zahiri M, Allahyari H, Esmaeilzade B. Proliferation of Human Spermatogonial Stem Cells on Optimized PCL/gelatin Nanofibrous Scaffolds. Andrologia (2022) e14380. doi: 10.1111/and.14380
107. Dash TK, Konkimalla VB. Poly-Є-Caprolactone Based Formulations for Drug Delivery and Tissue Engineering: A Review. J Controlled Release (2012) 158:15–33.
108. Shetty G, Mitchell JM, Lam TNA, Phan TT, Zhang J, Tailor RC, et al. Postpubertal Spermatogonial Stem Cell Transplantation Restores Functional Sperm Production in Rhesus Monkeys Irradiated Before and After Puberty. Andrology (2021) 9:1603–16.
109. Gaur M, Ramathal C, Reijo Pera RA, Turek PJ, John CM. Isolation of Human Testicular Cells and Co-Culture With Embryonic Stem Cells. Reproduction (2018) 155:153–66.
Keywords: spermatogonia, spermatogenesis, in vivo, in vitro, stem cell, 3D culture, male infertility
Citation: Diao L, Turek PJ, John CM, Fang F and Reijo Pera RA (2022) Roles of Spermatogonial Stem Cells in Spermatogenesis and Fertility Restoration. Front. Endocrinol. 13:895528. doi: 10.3389/fendo.2022.895528
Received: 14 March 2022; Accepted: 31 March 2022;
Published: 12 May 2022.
Edited by:
Barry Zirkin, Johns Hopkins University, United StatesReviewed by:
F. Kent Hamra, University of Texas Southwestern Medical Center, United StatesMichael Griswold, Washington State University, United States
Copyright © 2022 Diao, Turek, John, Fang and Reijo Pera. This is an open-access article distributed under the terms of the Creative Commons Attribution License (CC BY). The use, distribution or reproduction in other forums is permitted, provided the original author(s) and the copyright owner(s) are credited and that the original publication in this journal is cited, in accordance with accepted academic practice. No use, distribution or reproduction is permitted which does not comply with these terms.
*Correspondence: Fang Fang, fangfang0724@gmail.com; Renee A. Reijo Pera, reneer@mclaughlinresearch.org